Introduction
From the first wireless transmissions, electromagnetic interference (EMI) has been a concern for design engineers. The earliest spark-gap transmitters had no facility for spectrum control, and the presence of two transmitters in proximity caused interference in the receivers. These first transmissions were ‘one-to-one’ in that a single transmitter was communicating with a single receiver, and the information was in digital form, that is, Morse code using on-off keying (OOK). As more companies entered the field of wireless communications, regulation of the bands used was accomplished by negotiation between these competitors. This led to more efficient modulation techniques, frequency allocations and receivers with better selectivity. As electronics progressed, it became possible to send voice and video in analog form, and the transmissions became one-tomany broadcasts. This led to the need both to partition the broadcast bands for exclusive use by licensed carriers, and the need to regulate devices that might interfere with these transmissions. Now we have come full-circle: transmissions are once again digital in nature, and may be required to manage their own interference, as is the case in ultrawideband (UWB) systems and the many proliferating systems in the unlicensed Industrial, Scientific and Medical (ISM) band including Bluetooth, WLAN and others. In the licensed bands, we have cellular, satellite, broadcast and other systems creating a complex, dynamic spectrum environment. At the same time, other systems, such as computer, electronic and electrical machinery have exploded in popularity, increasing the probability of interference.
Regulatory agencies have placed limits on EMI levels, and have defined measurement methods for compliance testing. These methods have been in place for decades, and were written to accommodate the needs of analog broadcast of voice and video, and the test methodology available at the time of their writing. Examples of this are the CISPR averaging method and the Quasi-Peak detector. These measurement techniques are intended to achieve an acceptable interference level to the human ear and eye for audio and video respectively. With the emergence of digitally modulated data transmissions and ultra-wideband (UWB) transmission methods, combined with increasing frequencies of unintended radiators in the form of high speed digital clocks, the current standards of EMI compliance do not fully address all of the types of interference present today and their effect on communications systems. For example, an infrequent interference of short duration can be relatively high in instantaneous amplitude, but still meet the compliance regulations if it does not occur frequently. Such a pulse would have negligible effect on a broadcast analog radio transmission, but could cause the loss of an entire packet of data in a digital system, or jam an adjacent radar system.
Infrequent short bursts of interference have become much more common in all consumer electronics and communications. Examples include mode-dependent, spreadspectrum clocks used in computers, and hard disk drives that make periodic and noisy hard drive access cycles in many embedded system designs. Increasingly, these sophisticated digital devices are combined in proximity to wireless communications that are operating in a frequency-agile, packet-based mode. Consider a laptop computer or smart phone (Figure 1) that contains all of the high speed digital systems necessary in a sophisticated digital computer or phone combined with the wireless transmitters and receivers necessary for ubiquitous connectivity. The proximity of these unintended radiators combined with sensitive receivers is rife with interference opportunities, as seen in the tables of unintentional radiation sources and receiver bands in Figure 1.
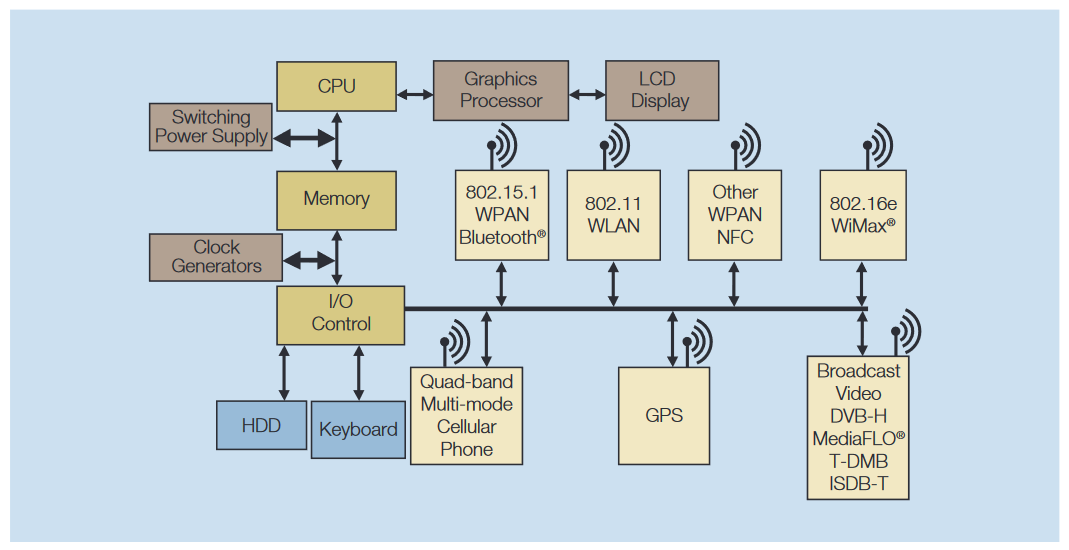
Typical Clock Sources | |
CPU | 200+ MHz |
Memory | 200+ MHz |
I/O Controller | 133 MHz |
Switching Power Supply | 400 kHz |
Graphics Processor | 200+ MHz |
Typical TX/RX Systems and Their Receive Bandwidths | ||
RF Receiver Technology | RF Carrier | Rx Bandwidth |
Quad-band cell phone | 800/900/1800/1900/2100 MHz | 200 kHz or 5 MHz |
Bluetooth | 2.4 GHz | 1 MHz |
WLAN | 2.4 GHz or 5-6 GHz | 20 MHz to 40 MHz |
WiMax | 2.3/2.5/3.5/4.9/5.0 GHz | 1.25/5/10/20 MHz |
Broadcast Video | ~200/470/700/1400/1600 MHz | 1.5 or 6-8 MHz |
GPS | 1.5 GHz | 1 MHz |
NFC | 13 MHz | Up to 2 MHz |
As the nature of interference in communications systems has changed, so has test equipment. Functions previously implemented with analog circuitry can now be done digitally, with increased measurement speed for faster results. Tektronix Real-Time Spectrum Analyzers (RTSA) can now view wide spans of spectrum instantaneously, with no lost information in the band. This allows discovery, capture and measurement of transient peaks that are very challenging to legacy techniques.
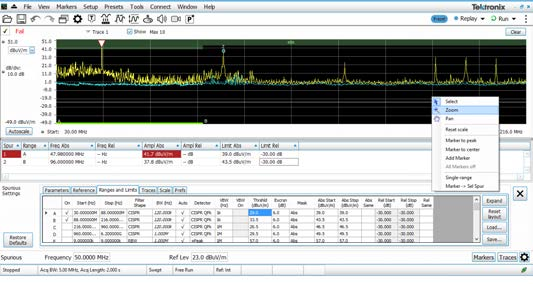
Diagnostics, Pre-Compliance and Compliance
In the world of Electromagnetic Compatibility (EMC), different equipment and techniques are used at different stages of design and qualification. At the early stages of development, design-for- EMC techniques are combined with diagnostics to produce low EMI signatures and low susceptibility to both external and internal interference. General-purpose spectrum analyzers with appropriate filters and detectors are often used to determine the effect of design optimizations for EMC. Probing is frequently done directly on the circuit board or with E-field and H-field probes to determine the effects of design optimization and shielding effectiveness. Of course, diagnostics are not limited to ensuring good EMC performance; system integration frequently requires extensive diagnosis and troubleshooting to ensure that all of the RF subsystems perform up to their required levels without being degraded by other parts of the integrated system. Pre-compliance testing is done after system integration to determine any problem areas in the design. Pre-compliance testing is not required to conform to international standards; the goal is to uncover potential problems and reduce risk of failure at the compliance test stage. The equipment used can be noncompliant and have lower accuracy and dynamic range than compliant receivers if sufficient margin is applied to the test results. Pre-compliance testing may be done in a certified lab using fast measurement techniques intended to give a ‘quick look’ at problem areas, or done at a temporary site by engineering personnel. Generalpurpose spectrum analyzers that contain appropriate filters and detectors are often employed in precertification, as they are fast measurement tools that often are already used in the design process and do not require additional capital expense.
If problems are uncovered at this stage, further diagnosis and design modifications are required. The features available on Tektronix Real-Time Spectrum Analyzers allow for some pre-compliance measurements in addition to diagnostics. An example of a pre-compliance scan is shown in Figure 2, combining the CISPR QP detected trace with an antenna correction table and the spurious search function. In this case, the trace is an ‘ambient scan’ looking at background signals present without a device under test.
Compliance testing requires methods, equipment and measurement sites in compliance with international standards.Compliance tests are commonly done as part of the design qualification prior to production of a device. Compliance testing is exhaustive and time consuming, and a failure in EMC at this stage of product development can cause expensive redesign and product introduction delays.
Filters, Detectors, and Averaging
Receivers and spectrum analyzers can be modeled as having a receiver bandwidth, a method of signal detection,and a method of averaging results to achieve signal level measurements.
In the case of many commercial EMI measurements, these measurement elements are defined by the Comite International Special des Perturbations Radioelectriques (CISPR), a technical organization within the International Electrotechnical Commission (IEC), an international standards body. Other standards and certification bodies, such as TELEC in Japan, also have requirements for measurement methods and certification techniques. In the US, the Department of Defense has developed the MIL-STD 461 with special requirements for military equipment.
The bandwidth of the measurement is defined by a receiver bandwidth shape or a resolution bandwidth (RBW) filter in the case of a spectrum analyzer. The bandwidths used are representative of the perceived threats within the spectrum, and the bandwidths vary with the receive frequency. The CISPR and MIL-STD filter shapes are discussed in this application note.
A detector is used to calculate a single point that represents the signal at an instant in time. Detection methods can calculate the positive or negative peak, the RMS or average value of voltage, or in the case of many EMI measurements, the QuasiPeak (QP) value. QP detection is explained in detail in this application note.
Averaging methods are applied to the detected signal over time. Averaging as defined by the CISPR standard is intended to reproduce the effect of reading the value of the signal with a voltmeter with a defined response time. Averaging may also be performed with a ‘video filter’ of a specified bandwidth applied to the detected output. For EMI testing, video filtering is specified in the TELEC standard. Both CISPR averaging and video filters are discussed in this application note.
Frequency Range | Bandwidth (6 dB) | Reference BW |
9 kHz to 150 kHz(Band A) | 100 Hz to 300 Hz | 200 Hz |
0.15 MHz to 30 MHz(Band B) | 8 kHz to 10 kHz | 9 kHz |
30 MHz to 1000 MHz(Bands C and D) | 100 kHz to 500 kHz | 120 kHz |
1 GHz to 18 GHz(Band E) | 300 kHz to 2 MHz | 1 MHz |
Table 1. Measurement Bandwidth versus Frequency specified by CISPR 16-1-1.
Filter Definitions
The level measured by a receiver or spectrum analyzer of any non-continuous signal will depend upon the measurement bandwidth used. To achieve consistent results, regulatory agencies have defined the bandwidth and shape of the filters used in compliance measurements. In the case of CISPR, bandwidths for Peak, RMS and average detectors are defined as seen in Table 1 and the shape of the filters are also defined in CISPR16-1-1. ANSI (American National Standards Institute), CISPR and MIL-STD 461E filters are defined by their - 6 dB bandwidths, while spectrum analyzer resolution bandwidths are traditionally specified at -3 dB. Resolution bandwidths of spectrum analyzers were historically defined as the separation required between two CW signals of equal amplitude necessary to create a just-visible ‘dip’ in the spectrum display, and so 3 dB bandwidths are specified. Spectrum analyzers designed for use in EMI applications will have -6 dB filter definitions as a user-selected setting (See Figure 4). The effect of using the -3 dB filter definition as opposed to the -6 dB definition can be significant. For the approximately Gaussianshaped filters with shape factor of 4.1:1 used in the RTSA, a 100 kHz filter specified at -6 dB will be only 71 kHz wide when measured at the -3 dB point.
The difference in random noise power measured will be 10*log10 (71/100), or approximately -1.49 dB compared to the traditional 100 kHz filter specified at – 3 dB, as seen in Figure 4. Using a spectrum analyzer with -3 dB RBW specifications will result in higher random and impulse noise measurements than with the EMI-specified filters, at the same RBW Value.
Frequency Range | Bandwidth (6 dB) |
10 Hz-20 kHz | 10, 100, and 1000 Hz |
10-150 kHz | 1 and 10 kHz |
150 kHz-30 MHz | 1 and 10 kHz |
30 MHz-1 GHz | 10 and 100 kHz |
1-40 GHz | 0.1, 1.0 and 10 MHz |
Table 2. Bandwidths versus frequency specified for peak, average and RMS detectors by ANSI C63.2.
Frequency Range | Bandwidth (6 dB) |
30 Hz – 1 kHz | 10 Hz |
1 kHz-10 kHz | 100 Hz |
10 kHz-150 kHz | 1 kHz |
150 kHz-30 MHz | 10 kHz |
30 MH-1 GHz | 100 kHz |
Above 1 GHz | 1 MHz |
Table 3. Bandwidths versus Frequency specified by Mil-STD-461.
The American National Standards Institute (ANSI) and the MIL-STD461 also define filter bandwidths for their measurements. The bandwidths chosen vary with the measurement frequency, as shown in Tables 2 and 3.
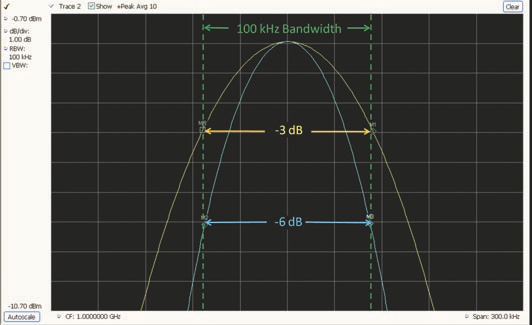
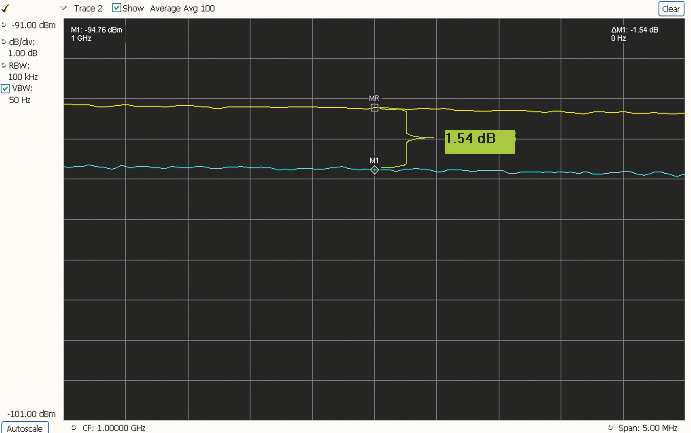
Characteristics | 9 kHz to 150 kHz (Band A) | 0.15 MHz to 30 MHz (Band B) | 30 MHz to 1000 MHz (Bands C and D) |
Bandwidth (6 dB) | 0.2 kHz | 9 kHz | 120 kHz |
Detector charge time | 45 ms | 1 ms | 1 ms |
Detector discharge time | 500 ms | 160 ms | 550 ms |
Time constant of critically damped meter | 160 ms | 160 ms | 100 ms |
Table 4. Characteristic of quasi-peak detector versus frequency specified in CISPR 16-1-1 and ANSI C63.2.
Detection Methods
While many EMI measurements can be made with simple peak detectors, EMI measurement standards have defined a special detection method, the quasi-peak (QP) detector. The QP detector serves to detect the weighted peak value (quasi-peak) of the envelope of a signal. It weights signals depending upon their duration and repetition rate. The QP detector has the characteristic of a fast-attack, slow-decay response and contains a time constant representative of a critically damped meter, as defined in Table 4. Signals that occur more frequently will result in a higher QP measurement than infrequent impulses.
Quasi-peak detectors have traditionally been constructed in the analog design shown in Figure 5.

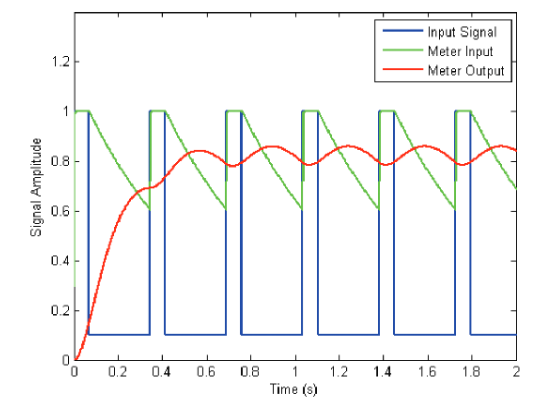

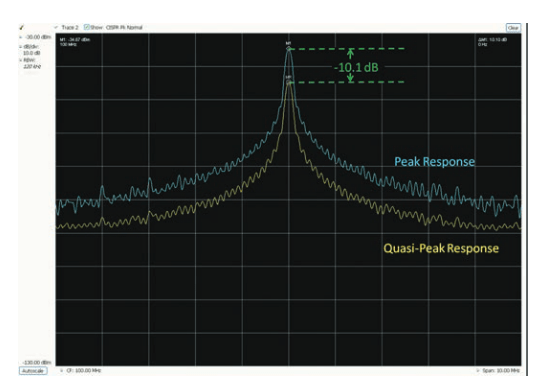
In Figure 5, the envelope of the signal Sin charges the capacitor C through resistor R1, as long as Sin is above S1. If the input signal Sin is less than S1, the voltage S1 is discharged by resistor R2.
To help visualize the response of the combination of the quasipeak detector and the associated meter, Figure 6 separates the input response (a repetitive pulse, seen in blue), the resultant quasi-peak detector response, exhibiting the fastattack, slow-decay characteristic in green, and the combined response of the detector and the meter in red.
For a constant indication on a receiver with a QP detector,the relationship between amplitude and repetition frequency described by CISPR 16-1-1 standard is shown in Figure 7.
An example of peak and QP detection is seen in Figure 8. Here, a signal with an 8 μs pulse width and 10 ms repetition rate is seen in both peak and QP detection. The resultant QP value is 10.1 dB lower than the peak value. When measuring EMI from a device under test, it is common to measure peak values first, to find problem areas that exceed or are close to the specified limits. Quasi-peak measurements, which are by nature slow, are then made only on the signals that approach or exceed the limits. Spectrum analyzers with standard peak detectors are often used to get a quick assessment of any problem areas.
Standards | VBW Requirements | Analyzer VBW setting |
CISPR | VBW not used | Maximum value or disabled |
TELEC | VBW = RBW or VBW ≥ 3*RBW | VBW=RBW or disabled |
MIL | Greatest value or not used | Maximum value or disabled |
Table 5.Video bandwidth requirements specified for EMI measurements.
Averaging and Video Filters
In addition to QP detection, the real-time spectrum analyzer also supports the peak and average detectors defined in the CISPR specifications. The peak detector detects the peak value of the envelope of a signal. The average detector computes the average value of the envelope. The RTSA is capable of measuring QP, peak and average values simultaneously from the same input signal and provides unique insights into the signal nature of DUT.
Video filters are specified in some EMI measurements, and were the original method used in spectrum analyzers to reduce the effects of noise variations in measurements. The name video filter derives from the original implementation, when low-pass filters were placed between the detected output and the Y-axis analog drive input of the CRT on the spectrum analyzer. RTSAs and some modern spectrum analyzers use digital techniques to achieve this smoothing of the noise on the signal.
In most EMI measurement cases, video filters are specified to be either off, or the video filter is specified to be at least 3 times greater than the specified RBW of the measurement (see Table 5).
The purpose of specifying the video filter to be off (or at least greater than or equal to 3 times the RBW) is to eliminate the effect of the video filter on the detected signal. Figure 8 shows the effect of Video Bandwidth (VBW) as it varies in ratio compared to RBW. When VBW ≥ 3*RBW, or at 10*RBW (or disabled), the noise standard deviation remains at 5.4 dB. When VBW=RBW, as is the case in some sections of the TELEC specification, the noise variation is reduced to about 4.7 dB.

Digital Implementations of EMI Filters,Detectors and Averaging
For spectrum analyzers based on Discrete Frequency Transform (DFT) techniques, filtering can be performed digitally by applying a window function to discrete acquisition data. The acquisition size is defined by the bandwidth of the required filter. Given the same sampling frequency, more samples are required to achieve a smaller filter bandwidth.
A Kaiser window is used on the RTSA to emulate EMI filters.The magnitude of the frequency response of the window function determines the IF filter shape and must satisfy the limits of pass-band selectivity defined in CISPR 16-1-1.
In RTSAs, the Quasi-peak detector is implemented with digital filters. Digital filters such as an Infinite Impulse Response (IIR) filter can be used to emulate RC charge and discharge circuits used in the traditional EMI receivers. The critically damped meter can also be modeled as a 2nd-order digital IIR filter. The maximum of the displayed value on the meter is taken as the Quasi-peak detector value.
Video filters are implemented with an averaging technique on RTSAs. The number of averages used depends upon the video bandwidth selected and the RBW in use at the time of the measurement. When VBWs are used, the resultant analysis length of the measurement will be driven by the selected VBW and will be longer than if an RBW were used without a video bandwidth. The RTSA selects the number of averages to achieve good correlation to the noise-variation vs. the VBW/RBW curve seen in Figure 9.
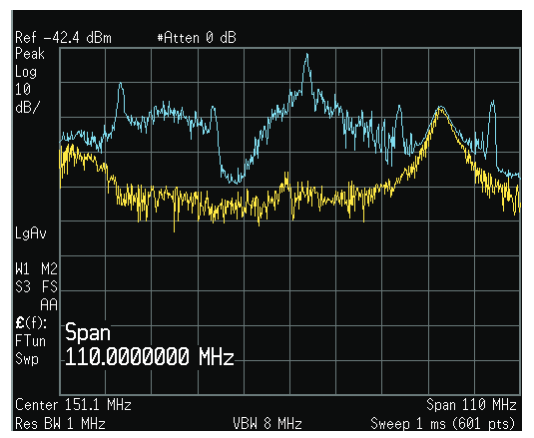
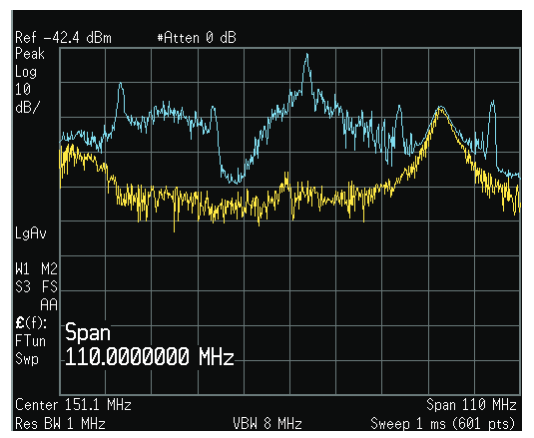
Measurement Speed and Real-Time Spectrum Analyzers
Measurement speed of QP and average values has been a continuing challenge for measurement receivers and spectrum analyzers. The long response time of the QP detector and meter makes it impractical to scan broad frequencies one at a time. To overcome this, measurements are made with peak detectors, quickly, to determine the highest peaks in the EMI from the device under test. Measurements are then repeated using QP detection on all problem areas, using single-frequency measurements. Recently, receivers and RTSAs have become available that can process large spans of information, applying QP detection and averaging orders of magnitude faster than single-frequency measurement techniques. This method of calculating all of the frequency points in the span yields significant speed advantages and has the advantage that transient signals in the band are seen with much higher probability of intercept when compared with swept techniques. This is especially important in today’s design environment, as signals change and move over time, and single frequency measurements are unable to represent these dynamically changing signals.
Troubleshooting Today’s EMI Problems
While the standards-based methods of measurement described above are necessary for regulatory compliance, they frequently do not address, or even detect, the problems faced in designing for EMI in today’s systems. The circa1930’s QP detector was not intended to determine the effects of today’s complex multiprocessor consumer electronics on the transient, hopping, digitally modulated and ultra-wideband signals used in modern communication and computing systems.
Fortunately, measurement techniques have evolved to match these needs. This section examines the use of the realtime spectrum analyzer to discover transient and hidden EMI problems, trigger on their presence, and analyze their characteristics.
In the example shown in Figures 10 and 11, a transient that occurred only during a single operating mode creates a series of transients that last for just a few seconds each time the mode is entered. In this case, the device is an embedded system, and the transient EMI is caused when the system is required to cache data to a hard disk drive. When briefly examined with the peak detector of a traditional non-real time spectrum analyzer (yellow trace, Figure 10), there appears to be just a continuous signal; leaving the instrument in Max-hold for several minutes while cycling the DUT operating mode indicates a problem (blue trace). However, making a fast scan in peak detect mode would have produced the yellow trace, and no indication of a problem is detected.
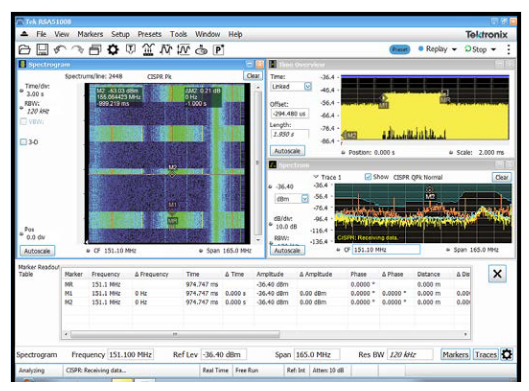
Examining the EMI signature of the DUT with Digital Phosphor Processing (DPX) in Figure 11 immediately discovers the problem. The DPX™ spectrum display, unique to Tektronix RTSAs, processes more than 10,000 spectrum measurements per second (exact number depends on which instrument in the portfolio is chosen), and ensures that any signal lasting longer than a few 10s of microseconds is instantaneously captured and displayed. More information on the operation of the DPX spectrum processor in RTSAs can be found in application note 37W-19638. The color grading seen in the display indicates frequency of occurrence of the signal. In the case of Figure 11, more frequently occurring signals are presented in red and infrequent signals in blue to green. It can be immediately seen which signals are continuous, and which are transient. The transient signal appears rarely, but at a level up to 40 dB above that of the continuous signal.
With a potential problem discovered by DPX, the next step is to trigger on and capture the signal for further analysis. This is easily accomplished by defining a frequency mask trigger based on the continuous signal profile, and capturing the infrequently occurring transient when it appears in the spectrum. Any signal above the frequency mask threshold lasting longer than the specified minimum signal duration will cause a trigger and store the pre-trigger and post-trigger signal into memory. The result of 4 acquisitions triggered by the transient is shown on the spectrogram on the left side of Figure 12.
Alternatively, in units equipped with advanced DPX, a trigger could have been set by simply right-clicking on the area of interest on the DPX spectrum display, and choosing ‘Trigger On This’ from the menu. Now the signal can be fully analyzed. The markers in Figure 12 show that the repetition rate of the transient is 1.0 seconds, but the length of the transient is not always the same. It varies from 752 μs down to 200 μs over the course of 4 acquisitions. This repetition frequency and varying pulse width were vital clues in identifying the source of the transient in the circuit- in this case it was diskcaching operations that only occurred under special operating conditions of the unit under test.
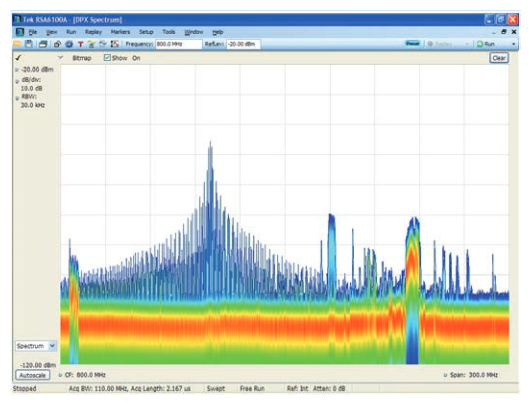
Using swept DPX spectrum analysis for troubleshooting.
In the example above, the DPX spectrum display was used within its real-time bandwidth. Tektronix RTSA can continuously process up to 800MHz span of spectrum (exact number depends on the instrument chosen), and signals longer than the specified minimum signal duration will be displayed on screen. When the required span exceeds the maximum real time bandwidth, DPX can still be used in a stepped fashion. For example, if a 300 MHz span is required, a 165MHz wide real time signal analyzer can step across the required band in several steps, and stitch together the resultant display. For stepped acquisitions the concept of minimum signal duration for 100% probability of intercept still applies, but only during the period of the sweep when an individual step is being processed. It is possible that the DPX display will miss transient signals that do not recur during the analysis time of the step
A technique for maximizing the probability of capture of infrequently occurring signals is to use the dwell time control of the DPX measurement. This control sets how long each step will dwell on its assigned bandwidth, and can be set from 50 ms to 100 sec. Consider a device under test that requires 5 seconds to go through a sequence of operations. Throughout the sequence, the radiated emissions of the DUT will change, and transients will occur that only repeat on the next sequence. The solution to guaranteeing capture of all transients during the cycle is to dwell the DPX display for a period longer than the sequence, and allow at least one full sequence to occur during each step. For example, a 300 MHz span that uses 100 MHz steps of the DPX spectrum analyzer would require 3 steps of 5 seconds each to guarantee capture of all signals in the sequence. A traditional spectrum analyzer may never see all of the transients, or may require an extremely long time to perform the analysis. A steppedsweep of 300 MHz is shown in Figure 13. The large pulsed signal shown appears at 5 second intervals, and lasts for 1 millisecond, occupying several hundred MHz of spectrum. It is captured on every ‘sweep’ of the analyzer in complete detail, because each step of the analyzer dwells for 5 seconds over the real time bandwidth.
Conclusions
Making EMI standards-based measurements requires special filters and detectors as defined by standards bodies. These special filters and detectors are available in receivers, conventional spectrum analyzers and Tektronix RTSAs. DSP techniques used in RTSAs and some receivers can make measurements orders of magnitudes faster than swept methods, because an entire real-time span (up to 800 MHz) can be analyzed simultaneously.
Real-time analysis greatly reduces the minimum signal duration required to guarantee 100% probability of intercept of transients compared to swept techniques. Troubleshooting systems that create a broad variety of transient signals can be most easily performed with a RTSA that has DPX spectrum processing, ensuring that no transient is missed during the analysis. This improves time to insight and creates higher confidence in the quality of the device under test.
Find more valuable resources at TEK.COM
Copyright © Tektronix. All rights reserved. Tektronix products are covered by U.S. and foreign patents, issued and pending. Information in this publication supersedes that in all previously published material. Specification and price change privileges reserved. TEKTRONIX and TEK are registered trademarks of Tektronix, Inc. All other trade names referenced are the service marks, trademarks or registered trademarks of their respective companies.
01/16 37W-22084-2